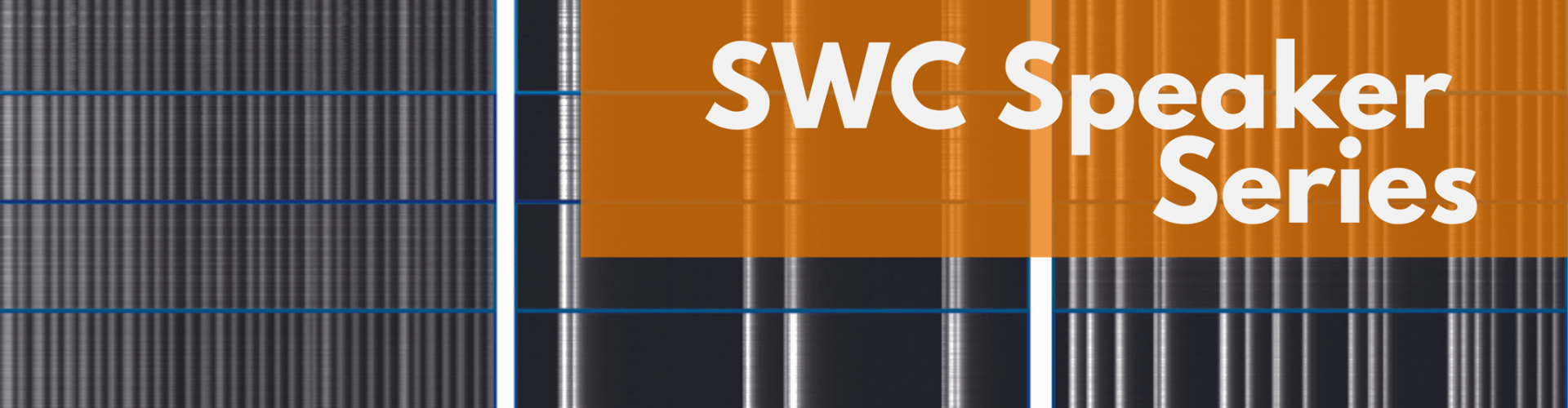
What can cortical layer 5 teach us about unconsciousness and development?
An interview with Drs Arjun Bharioke and Martin Munz, Institute of Molecular and Clinical Ophthalmology at Basel, conducted by April Cashin-Garbutt
In the last lecture of the 2021 Emerging Neuroscientists Seminar Series, Drs Arjun Bharioke and Martin Munz shared their work exploring the neural response of cortical layer 5 to different anaesthetics and the role of synchrony in consciousness and development. In this Q&A, they elaborate on the use of anaesthetics as a powerful toolkit for studying cortical circuit function and formation, their key findings so far and the next pieces of the puzzle their research will explore.
What makes cortical layer 5 unique? How does it compare to other cortical layers?
Dr Martin Munz (MM): Cortical layer 5 has a higher degree of recurrent connections than other layers. Just like layer 6, layer 5 is an output layer of cortex, but its subcortical outputs go to higher-order thalamic regions. This means that layer 5 is perfectly placed to interrupt information flow because it’s an output layer to higher-order areas.
Dr Arjun Bharioke (AB): The architecture of cortical layer 5 is convergence and then divergence. Information from many inputs converges onto layer 5 neurons through their huge dendritic arbors. It also receives input from lots of other layer 5 neurons that each receive information from their local region as well. So there are these large regions of convergence from different areas, which are then followed by divergent transmission of information downstream to other cortical areas as well as other subcortical areas.
If one was looking to shut off the system, one would want to shut it off exactly at this point. You can think of layer 5 as a bottleneck in the cortical circuit, partly because of its recurrence that gathers all these inputs in, and partly because of its architecture that sends their projections out to interesting areas. That, to me, is what makes layer 5 particularly unique.
MM: Along with many people around the world, we are still in the process of answering this question. However, we are not dedicated to being a fan of layer 5! The questions we are trying to answer apply not just to layer 5, but to every single layer.
AB: We didn’t come into this research thinking that layer 5 was going to be the layer to look for. We came in with a more naïve approach of not knowing what’s happening within cortex. In our case, we found something interesting happening within layer 5 and then went to look at the other layers. Then, we found that this phenomenon is actually isolated solely to layer 5. We, then, went to think about why that might be the case.
Previously, researchers like Michael Hausser and Tiago Branco identified the apical dendrite of layer 5 pyramidal neurons as a unique structure, and explored many of the computational properties introduced by its presence. It’s fascinating to us that we happened across a network effect specifically in layer 5, with all of its fascinating single-cell properties.
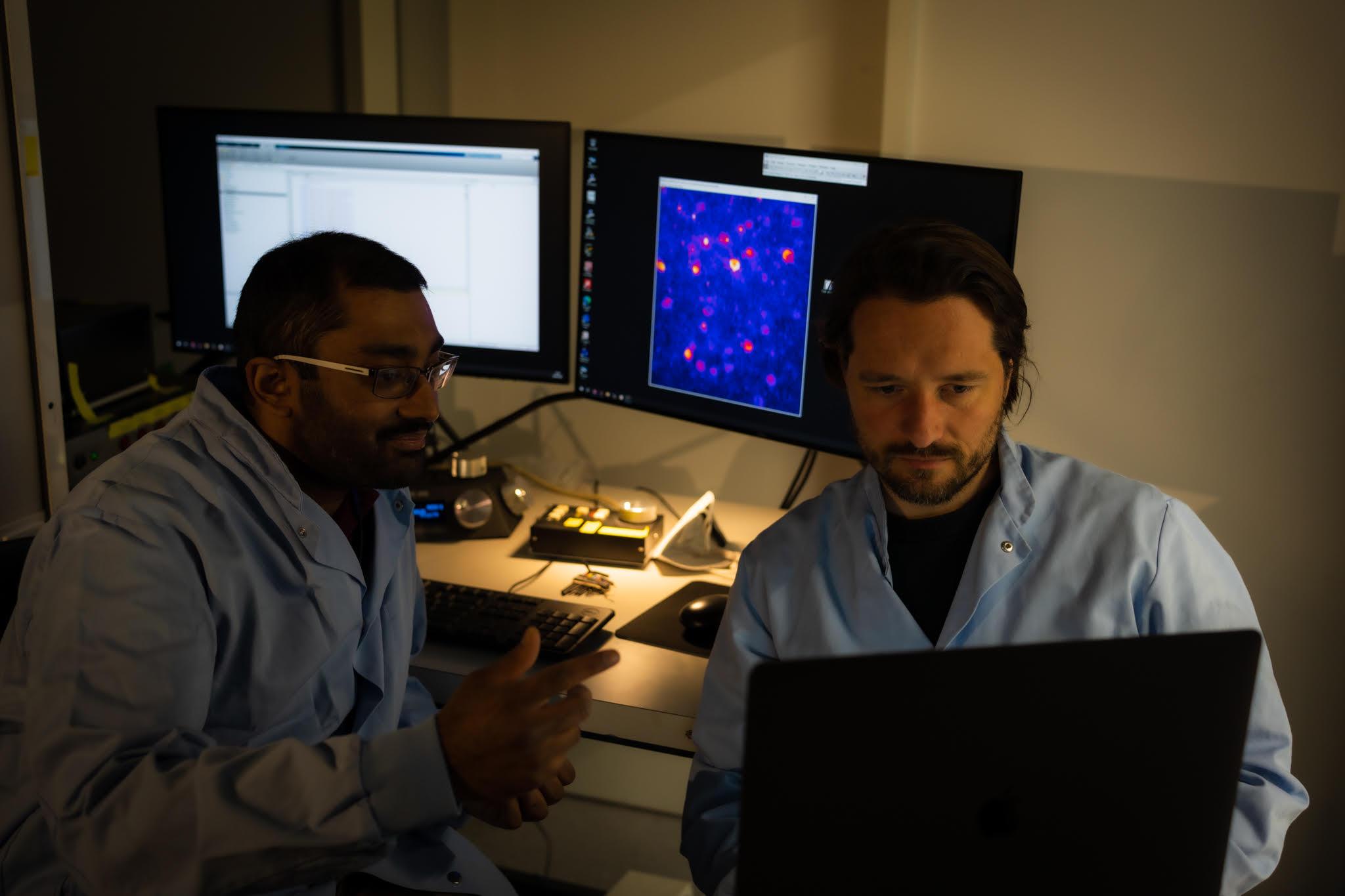
Why does your research focus on cortical layer 5 during early development and unconsciousness?
MM: Development is an interesting period of time because you can see which parts are built first and which parts built later. We are very interested in what happens in an adult cortical circuit, but also during development because when approached from both directions there might be a better understanding. With your car, for example, you can know how to drive it but if you also know how it’s built, you have a better understanding.
AB: Layer 5 is a recurrent network and we know that recurrent neural networks (RNNs) are extremely powerful, and can perform lots of interesting computational tasks. Training an RNN network is quite different than training other deep networks (convolutional neural networks, for instance) that people had used previously. Perhaps by understanding how the brain makes layer 5, its own biological recurrent neural network, we can gain insight into how we could apply this to artificial neural networks.
Why did you induce unconsciousness using anaesthesia to study the function of layer 5?
AB: To me, the beauty of anaesthetics is that they provide a very repeatable and rapid transition of the cognitive state of an animal. Two ways to induce unconsciousness are sleep and anaesthesia. What happens during sleep is a fascinating question that we are also very interested in, but sleep is a much more variable thing to work with. For example, in mice you induce sleep by keeping them awake for a very long time, after which they fall asleep. In contrast, with general anaesthestics, you really can apply exactly the same protocol repeatedly and you get the same result. Further, the exact same relative dosages of anaesthetics (adjusted only for the weight of the organism) result in anaesthesia in lots of species, from humans all the way down to reptiles. For example, you can use the same percentage of isoflurane to anesthetise a reptile, bird, and a human. The beauty of this is that if you understand anaesthesia better in one system, this is the rare situation in biology where it may apply across an entire kingdom. This makes studying anaesthesia particularly appealing.
Another aspect that is appealing to me personally is that anaesthetics act faster and more powerfully than you might imagine. You inject or just inhale a drug, and in 35 seconds to a minute, the animal is completely anaesthetised – its qualitative behaviour completely changes in such a short time. For an inhalation anesthetic, the drug has to go into the lungs, the bloodstream, cross the blood brain barrier, and then affect something in the brain. Yet, this all happens within seconds.
Further, we have multiple different anaesthetics, all of which affect completely different molecular systems. And yet, somehow, they all result in the same convergent effect on the organism. Therefore, anaesthetics provide a simple yet beautifully powerful toolset that allows us to manipulate the dynamical system of the brain. It is just like any other manipulation such as optogenetics, but in this case it is a pharmacological manipulation that is rapid, repeatable, and controllable. To me, that’s the power of the system.
In what ways did the activity of layer 5 neurons change across different anaesthetics?
MM: We found that, upon administration of different anaesthetics, activity changes in different ways across neurons, with some similarities, and some differences. The activity of pyramidal neurons within cortical layer 5 seems to align. However, how often the cells are active is different for different anaesthetics. For example, in isoflurane, they are active every few seconds. On the other hand, ketamine produces activity every second or so.
In this particular project, we focused on the aspects of anaesthesia that are the same between different anaesthetics. But in the future, we also want to explore the differences between different anaesthetics.
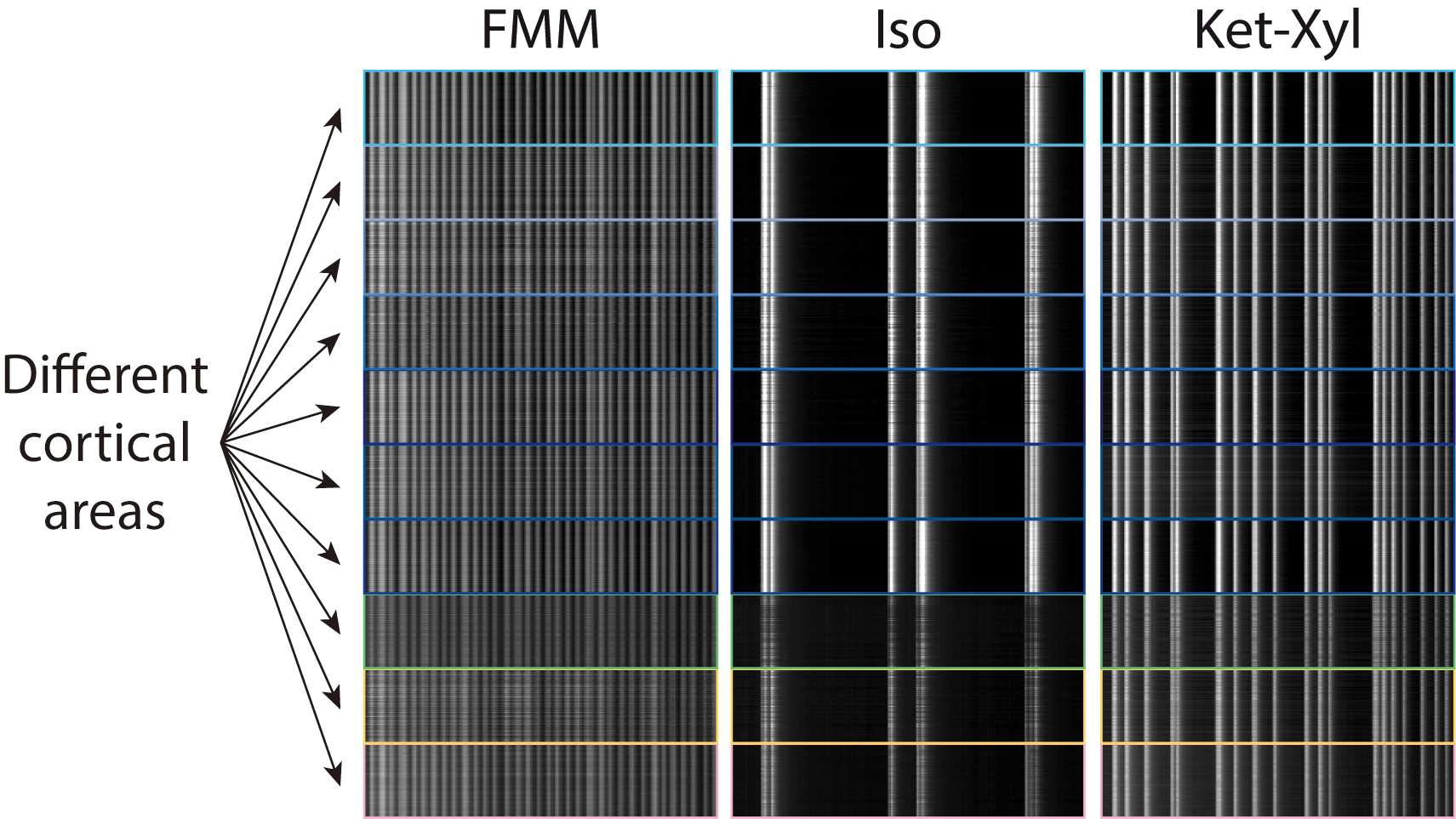
AB: If you also go beyond layer 5 to other cortical layers, there you will again find big differences across anaesthetics. There, the differences outweigh the similarities. In ketamine, you see synchrony in layers 2/3 and 4 as well as layer 5. But you don’t see this with fentanyl-midazolam-medetomidine (FMM). In particular, there is no synchrony in layers 2/3 and 4. We know, from work in humans, that ketamine produces what is known as a dissociative state, and can also cause hallucinations, so there are potentially interesting correlations of the differences in activity across cortex with the behavioural effect of these anaesthetics.
This really emphasizes another advantage of researching anaesthetics – since anaesthetics work across species, you can actually ask a human to report on their experiences, whereas you can study the mechanism in the mouse.
How does the synchrony in layer 5 during anaesthesia compare to during sleep?
MM: This is definitely one of the things we want to explore in the future. Sleep and anaesthesia clearly have some similarities, but there are also big differences. You don’t come out of anaesthesia feeling refreshed, instead you’re usually confused. We want to study not just the similarities, but also the differences.
AB: With sleep, you have multiples stages. So it’s possible that anaesthesia is actually similar to one stage of sleep. One difference from anaesthesia could be that with sleep you nicely transition from one stage to another and wake up at one particular stage. Maybe that is why when someone wakes you up in the middle of a sleep cycle, you are much more groggy and confused. We haven’t explored sleep at all, and that is an exciting path we’d like to explore.
Were you surprised to find changes in layer 5 synchrony coincided with the loss and return of consciousness?
AB: We didn’t expect them to match so nicely. We did see that there was always synchrony with the onset of anaesthesia, and we knew that it was present at some point, but the idea that the timing of the neural activity matched was fairly surprising.
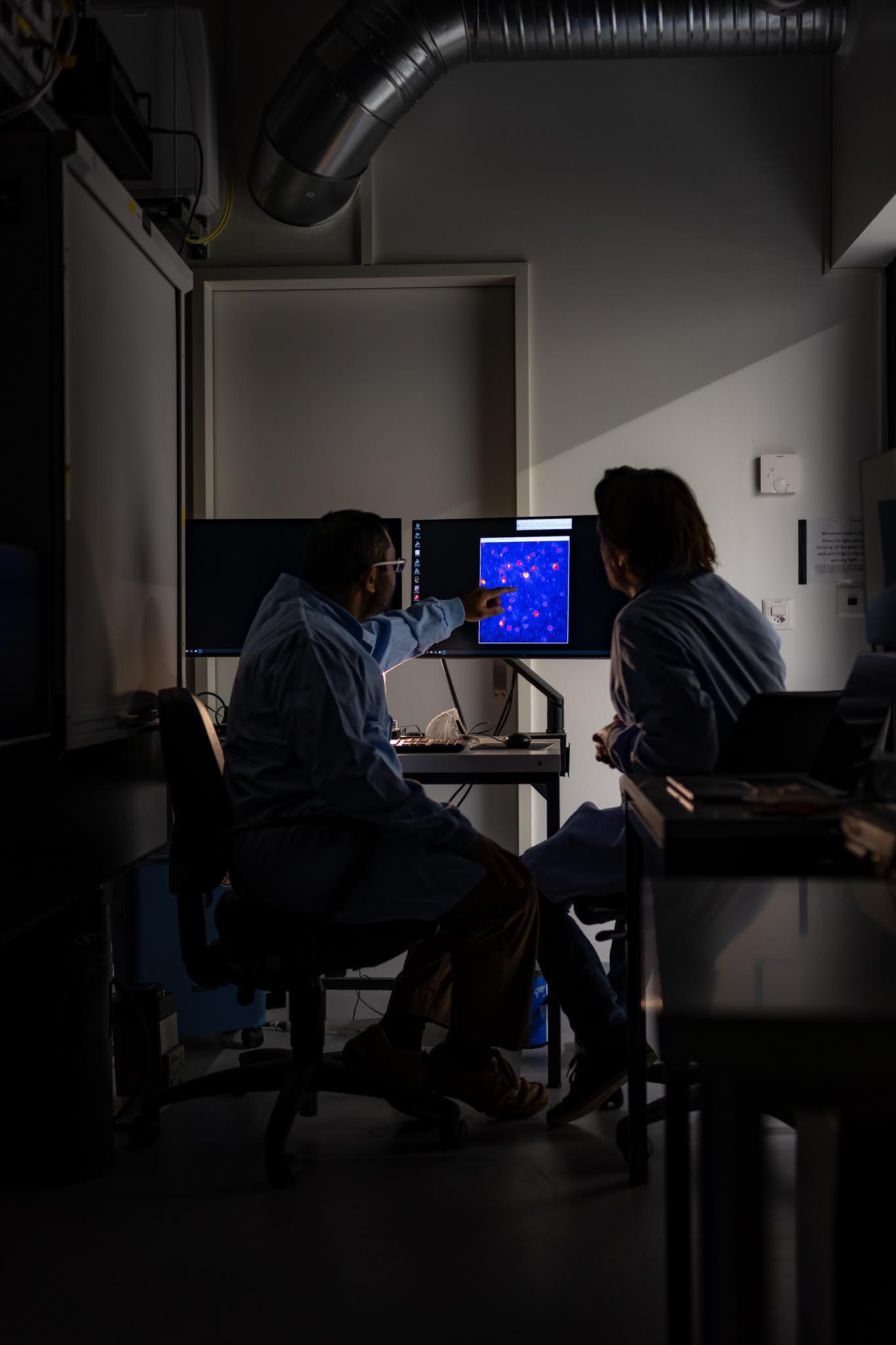
How do you study the development of layer 5?
MM: Here the idea is to go to the very beginning of cortical circuit development. A lot of research has been done trying to understand how neurons connect on various levels, including the molecular level. We wanted to go to the very beginning of when neurons first appear in the neocortex, and see what happens at a circuit level. We found that these neurons are active very early on, which was quite surprising. Also, this activity did not just ramp up over development, but instead changed in a structured way.
AB: If you want to go the beginning, the whole cortex actually develops from layer 6, then 5, then 4, and so on – basically, it develops in reverse order. So, we knew we had to go to one of the earliest developing layers.
What implications does this research have more broadly for understanding unconsciousness and development?
MM: In terms of development, the questions we are asking are really basic science ones. What we are trying to understand here is how pyramidal neurons, which are so fundamental to cortex, form a circuit.
Then one might ask, how does it help humanity? There are quite a lot of diseases that involve defects during development, called neurodevelopmental disorders. One example is autism, but many others as well, including schizophrenia and epilepsy. To understand these disorders, our approach is to understand what happens normally. With that understanding, one could then observe the differences from normal development when a particular gene associated with autism is manipulated.
For now, we are really laying the groundwork to understand the development – which personally I find fascinating in and of itself – and next we want to extend that understanding to what happens when things go wrong.
AB: Our logic of understanding development is understanding circuit development specifically. Most neurodevelopment disorders are thought to be disorders of circuit dysfunction. In neuroscience, we have focused on diseases that are the result of single gene mutations. One gene changes and a disease develops. In contrast, many neurodevelopmental disorders result from the combination of mutations across multiple genes. However, these diseases do result in overall changes to the circuit structure. For example, in autism, there are changes in synaptic density. As such, it may be helpful to study these diseases from the point of view of their circuit architecture. This drove our interest in the direction of our work, building understanding of the normal development of neural circuits.
MM: In terms of anaesthesia, the same principle holds. There is, on the basic research side, the question about how you change activity in a brain in a particular way, and how that produces unconsciousness.
Then, there are questions that impact people’s lives. There are lots of different things that change in our lifespan when we are exposed to anaesthetics. Both early on in life and late in life, anaesthetics are known to act differently. There are some interesting questions that revolve around how this might occur. Right now, we’ve laid the groundwork to know where we should be looking, and in the future we want to address more specifically what happens, at different ages, for example.
AB: Anaesthetics, from the basic research point of view, can also be viewed as a toolset. We want to understand dynamical systems and cortical circuits in the brain. If we want to understand that, we need to have good ways to drive dynamical systems, in order to study these systems in a repeatable way. And anaesthesia serves as a really powerful tool to do that.
On the more clinical side, it would be useful if we could potentially disentangle the functions of anaesthetics itself. When you anaesthetise someone, they lose muscle tone, change their breathing rate, change their heart rate, change their consciousness, and lose their memory of the period of time when they’re unconscious. It is not clear that each of those things has to be entangled with one another. I don’t think we fully understand what parts of them have to be entangled and what parts do not. So anaesthesia may potentially provide a circuit target for one part of this phenomenon which may not affect other parts. That would actually be very valuable for modern anaesthesiology, to titrate one symptom down, while maintaining some other function.
You have also studied a variety of other species/model systems including flies and frogs. Are there commonalities in the circuit mechanisms you have studied or any revealing differences?
AB: The bee has a really interesting circuit system. The bee has only 1,000,000 or so neurons in total. This is orders of magnitude less than our brain, and yet a bee can do really interesting tasks like navigating mazes. For example, in Mandyam Srinivasan’s lab, they have trained bees to navigate a maze by remembering the colour of a cue that they receive before entering the maze, and then following a different path, depending on the colour. Again, it is doing all this with a brain that is only 1,000,000 neurons in size!
The solution that organisms like flies, which I worked on in my PhD, or bees find at the circuit level may, however, be very different from what mammals do. The reason for that is often because of specialisation. In bees and flies, there are often extremely specialised circuits that do one particular task. You do have areas of the bee or fly brain which are associative and link many different things together. But you also have a lot of areas which are tuned or pared down to do the absolute minimum necessary to perform a particular task.
When studying these systems, it is interesting to ask, what is the fundamental necessary algorithm required to do this task? This applies to organisms that have constraints on the number of neurons.
In contrast, the solutions that our brains use are very different, and we have different neural constraints, so the organisation of the circuit is very different. But at the same time, the genetic architecture, organisation and development of these systems are often remarkably similar to each other despite the differences from a circuit architecture point of view. So that brings up interesting questions as to why there are these similarities on one hand (homeobox genes organising the brain), and yet the solutions for circuit architectures that they pick are significantly different.
MM: I think this is an interesting question for development because you could have a fixed blueprint of a brain, and that may be how you build each brain. However, in a world where things are not always the same, you want to have the possibility to change that depending on how you grow up or what happens during your development. So there are very interesting mechanisms that incorporate responses to the changing environment in the circuit.
For example, if you think about the computer, it always has the same circuit, but it can do different things if you put on a different software. The brain is different – the change is made down to the level of circuitry, depending on which task it performs. I think that is a super interesting question and is true in tadpoles, as well as mice. It is less true in Drosophila (fruit flies), where they use a different strategy where neuron A connects to neuron B, which certainly offers less flexibility. To me, this is an interesting question that can be nicely explored in different species.
What is the next piece of the puzzle your research is going to focus on?
MM: One thing that we are interested in on the developmental side is trying to get at the question of genes that are related to diseases like autism, schizophrenia, and other neurodevelopmental disorders of circuits. Additionally, we are interested in how activity impacts the development of circuits, specifically cortical circuits. It’s super interesting that there is already activity right at the initial formation of the six-layered cortex.
AB: At the time point of embryonic day 14 in mice, there are no external inputs into cortex. Thalamic axons have not even entered cortex. Retinal ganglion cells have not connected to thalamus. So in some sense, this circuit is forming in isolation. Why form a circuit in complete isolation? Why not just wait until there are inputs? Even if the purpose of inputs is to tune accordingly based on development, why have the circuit develop beforehand?
On the anaesthesia side, there are a lots of questions to ask! Sleep is a very interesting topic, and so is understanding how driven oscillation works in the neural circuit. For example, if there is a slinky that is connected to other things and the slinky starts to move, then after a while all the connected parts will be moving at the same rate that I shake the slinky. This is called driven oscillation.
In a system of connected things, if you drive one component at a set frequency, all are affected at the same frequency. But that is not what we see in layer 5 pyramidal neurons during anaesthesia. Instead, we see a non-oscillatory synchrony, that is the synchrony doesn’t follow a fixed rhythm. And that is a very hard thing to accomplish in a connected network. So really understanding how that could happen would give us important insight into how cortical circuits are different from a simple connected network. That would provide a lot of value for understanding how cortical circuits can generate functional activity.
Another fascinating aspect of the layer 5 circuitry is that there are different underlying neuron types, each with different arborisations. We are interested in better understanding the role of these distinct types in generating and maintaining the synchrony. A transistor is a transistor no matter where it sits within a computer chip. But a PT (pyramidal tract) neuron has a completely different arborization and projection size than an IT (intratelencephalic) neuron. The difference in structure strongly suggests that the specific identity of the neurons in the system matters. That provides some power to the neural system which we don’t fully understand just yet. This is a big advantage of real neurons over computer circuits. And studying the synchronous activity generated by anaesthesia gives a way to approach this question.
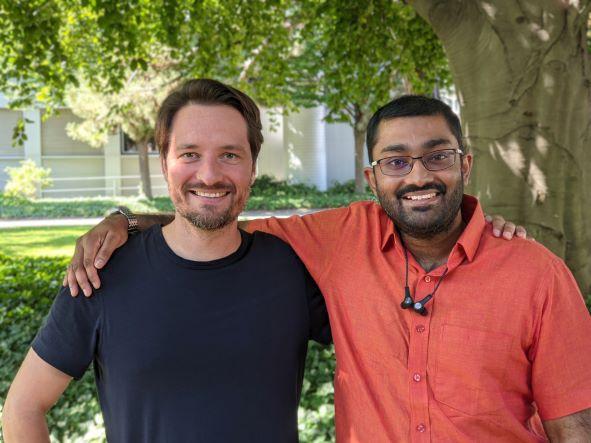
About Drs Arjun Bharioke and Martin Munz
Arjun Bharioke's interest is in the relationship between neural circuit architecture, activity, and function. After early experimental work exploring learned somatosensory behaviours (with Karel Svoboda, Janelia Research Campus), he transitioned to theory, and identified candidate neurons for the elementary motion detector within the Drosophila optic lobe connectome (with Mitya Chklovskii, Janelia Research Campus). In his postdoc, he decided to return to experiment, and joined the Roska lab to study cortical circuits. He became interested in the layer 5 circuitry, given its highly recurrent nature and the presence of spontaneously active dynamical states, making it a perfect testbed to ask deeper questions about architecture, dynamics, and function.
Martin Munz worked initially on natural somatosensory behaviours in the Etruscan shrew (with Michael Brecht, Bernstein Center for Computational Neuroscience Berlin), and became interested in understanding activity-dependent circuit development. By manipulating individual retinal ganglion cell axons within the Xenopus optic tectum, he showed how neuronal activity can modulate circuit architecture (with Edward Ruthazer, Montreal Neurological Institute). His interest in the Roska lab was to explore the role of activity in organizing the developing mouse cortex in the living embryo. Activity in early neuronal circuits is spontaneous, given the absence of sensory inputs. Hence, studying embryonic circuits provides another opening to explore spontaneous activity states within cortex.