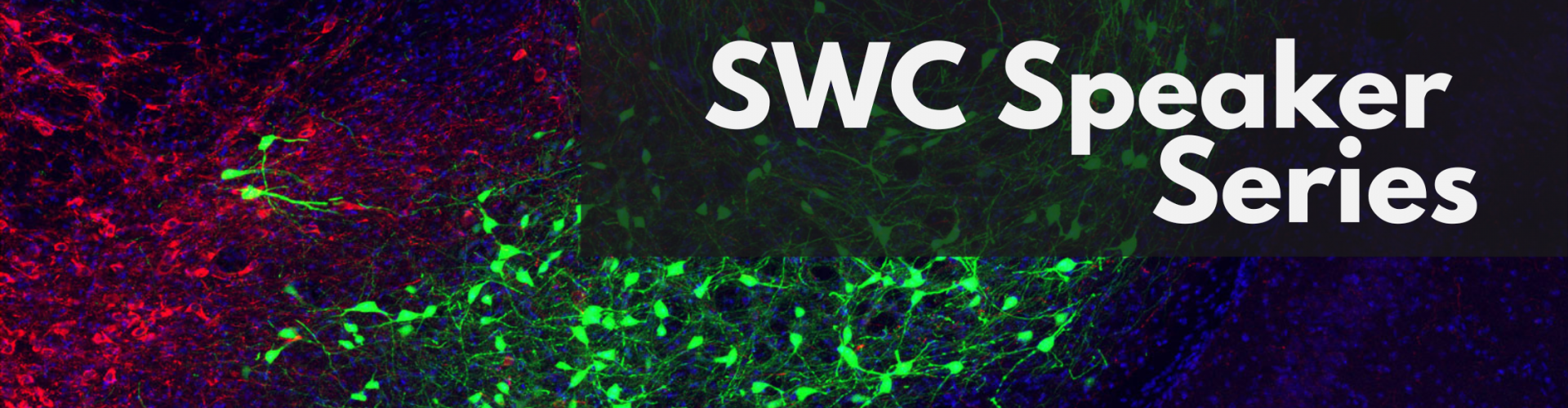
Controlling the order and timing of action sequences
An interview with Dr Xin Jin, East China Normal University, conducted by Hyewon Kim
From washing the dishes to typing in the password to log on to our devices, learned action sequences are integral parts of our lives. Each action sequence is made up of individual steps that are organised into the proper order and executed at the appropriate times. How does the brain control the order and timing of action sequences and what happens when this circuitry is disrupted? In a recent SWC Virtual Seminar, Dr Xin Jin shared his work exploring the neural mechanisms of action sequence order and timing. In this Q&A, he shares some of the backstory, next steps, and more.
Can you share some examples of actions that are organised into sequences? What is the advantage of sequencing and is it more common in complex behaviours?
If you think about our daily life, we are using computers, iPhones, inputting passwords for emails or ATMs all the time. Everything is an action sequence – we unconsciously learn and perform it every day. Adults drive cars, play sports, or kids play piano. My son plays the piano, which is a very good example: you have the action sequence, then the sound feedback. In fact, the sound feedback is considered a closed loop. It is a continuous loop of an action and the consequences of the action from the outside world. That’s actually consistent with the majority of our daily life.
Action sequences like breathing and walking are built into our genes through million years of evolution, into the circuitry you are born with. On the other hand, we have activities like the ones we just described, which are mostly learned, but are also considered action sequences.
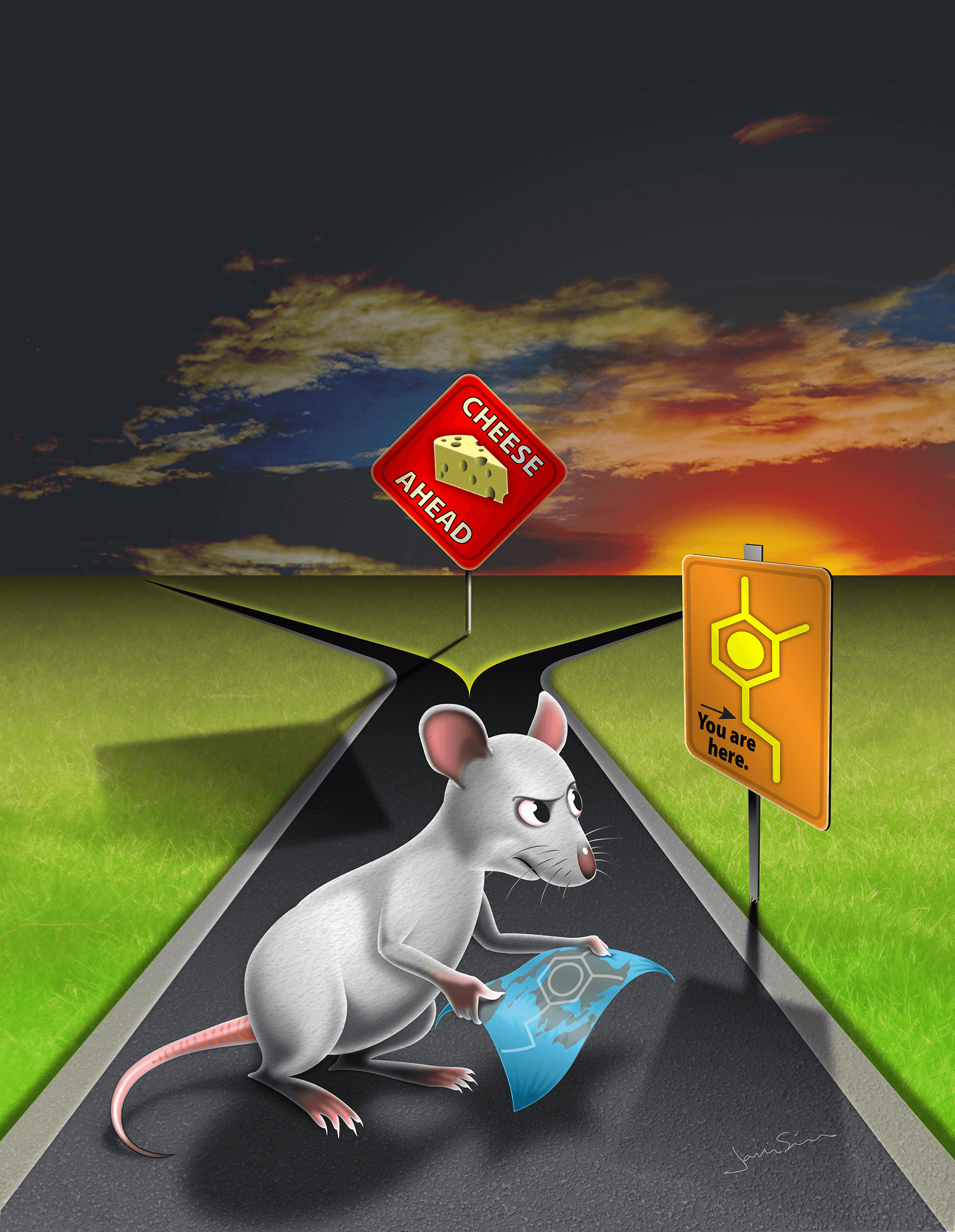
If you go further into the clinical side, the simplest example I can give to you is Parkinson’s disease. If you ask someone to draw a V, they can do it very easily. But for a Parkinson’s patient, they get stuck at the bottom angle for a long time. As soon as they start drawing, they can continue drawing the downward slope, but they cannot turn around.
The second well-known example is that when you learn to play the piano, the more you practice, the more quickly you can do it, and more efficiently. But a Parkinson’s disease patient, even after five or ten years, when they try to put their clothes on every morning, they still take the same amount of time as they had in the beginning.
So my argument, in terms of the clinical side, is that you have both problems of executing and learning an action sequence. A patient does not improve over time. So if you put all these three things together, you have an innate capability to do certain things and you have a capability to learn new action sequences, which we call skills. And on the clinical side you have people with Parkinson’s and kids with autism or OCD, who repeatedly do the same thing again and again, even if they want to do something else, they cannot finish the switch between different actions.
All this together suggests two things. Firstly, an action sequence is a fundamental part of our daily behaviour, and secondly, there are neurological and psychological diseases that show fundamental problems in organising an action into a proper sequence.
To generalise a bit more, the motor part is very obvious because that is just about doing. But through evolution, it became more and more advanced or sophisticated, meaning we now have an internalised action sequence. For example, you already planned to do an interview with me, but you actually planned three steps in your head. These things have not been executed yet. For me, to compare with you – since you are a professional, so you can do this planning in a second – I need a day to prepare. The difference with professional chess players or executives is that they can plan the action sequence much better than us amateurs.
These four points set the foundation. It’s important for neuroscience to understand how the brain organises and mediates these action sequences.
How much is already known about innate action sequences in relation to central pattern generators and what remains unknown?
We actually know quite a bit and that was one of the first major progresses people made in the motor control field many decades ago. Initially people believed that behaviour was driven by outside stimulation. It’s like you poke someone, and then they jump – that is how we used to think of the brain. And then, in the early phase of the motor control field including work done by pioneers like Erich von Holst, one major contribution made to neuroscience about how the brain works was the discovery in lower animals, from insects to fishes, that neurons in the central nervous system have intrinsic firing activities. The neurons were activated without external stimulation. So the initial theory was that maybe the brain can do something by itself. That is how the innate action sequence or motor control field began to flourish.
Work done by the early ethology school including two Nobel laureates Konrad Lorenz and Niko Tinbergen have further extended this idea. They studied how the egg-retrieval behaviour in gooses. For example, if the goose is performing the egg-rolling back sequence, once it initiated the first step back toward the egg, the researchers took out the egg from its spot in front of the goose. They discovered that the goose has no choice but to finish the remaining six steps as if the egg were there! They have to finish it before they do anything else. That is how people realised that the brain has a fixed pattern – geese have to finish the action sequence, with or without the actual egg, also known as an external object. As long as they initiate it, they have to finish it. For the rodent, there is the grooming behaviour, which is also a series of action sequences. This was what was known in the field, and mostly that the subcortical neural network including brain stem and spinal cord were responsible.
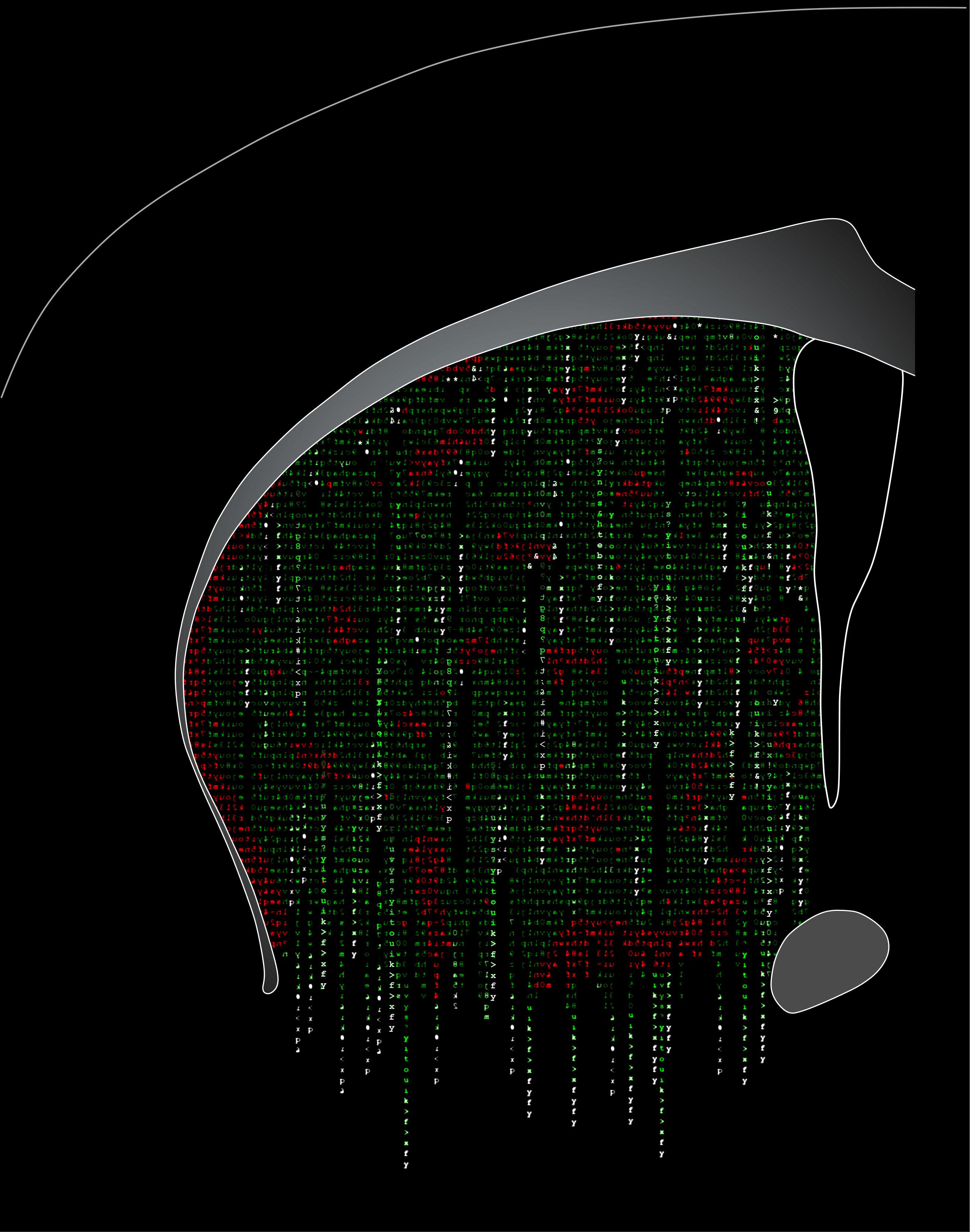
We still have a lot to learn about the central pattern generators in the mammalian brain. We do know quite a bit how the central pattern generator works for breathing. But for locomotion for instance, it is still far from a complete picture.
What did you do to try to find out how learned action sequences are controlled and organised into proper order and timing?
Firstly, the most difficult part for everybody in the field, not only for my research group, is studying behaviour. If you’re dealing with a human, that’s quite easy – you just see what happens in the brain when they play the piano, for example. But then the problem is that you do not get to see the firing activity of a single cell in the place you want. Traditionally, there were prominent neuroscience studies of the macaque, which made a huge contribution to the field in identifying the neuronal correlates of an action sequence – how the neurons fire. That gave us some idea into how single neurons or particular brain regions worked. But the challenge there is that in monkeys we lack the genetic tools to study aspects related to human disease, as well as manipulation tools to establish causality. For example, if you put an electrode in the monkey brain during the performance of action sequence, but it is not easy to establish which part of the network indeed controls or is necessary for the behaviour.
Due to all these limitations, the field is moving more towards using rodents, particularly mice, as an animal model. In mice, we have more genetic tools available, and due to their numbers it is a more efficient and reliable way to perform studies. However, the biggest challenge was, and continues to be the development of proper behavioural paradigm in mice. For instance, it took us many years to get the simple left-left-right-right ‘Penguin Dance’ action sequence task to work. Before that, we didn’t know what mice could or could not do. So there was a lot of trial and error, customised design both for the behaviour and environment. The behaviour was the biggest challenge, and we spent a lot of effort to develop a new variety of different tasks in mice targeting a specific question. Two examples, as I mentioned in the talk, are order and timing.
For order, we designed the tasks specifically to study switching behaviour. In fact, we have some preliminary results to share, that the autism or obsessive compulsive disorder (OCD) animal models do have a problem in performing or learning the task. This means we can establish a mouse model not only to study the neural mechanism, but also somehow to connect to the human. This is one of the biggest motivations for both studying behaviour and for the timing project. This first approach is about intelligent design and the hardware realisation of the task.
The second approach to study this behaviour was observing the brain of the animal exhibiting the behaviour. Observing traditionally meant you perform electrophysiology, which records from single neurons. In our case, we put an array of electrodes into the brain, thereby recording from tens of neurons. With new technologies, like the Neuropixels developed by a team including UCL, we can now record from hundreds to thousands of neurons in the brain of the behaving animal. You can then collect more information at the single neuron level and then connect populations of neurons across brain regions in the animal doing the task. You can monitor the whole network activity at single neuron level during the behaviour and then try to see how the neural population dynamics correlate with the behaviour.
On top of that, a major thing for us was that we wanted to know the cell type. Looking at a single brain region is not enough to study this because not every neuron is equal, like how we as humans have different personalities and jobs. We can therefore take advantage of mouse genetics and not only monitor the activity of neuronal populations, but also their molecular genetic identity. You can know if a certain neuron belongs to a genetic group. We can also use virology tools to sort out the input or output, which is the upstream or downstream of those neurons – in other words, who they listen and talk to, respectively. And of course complementarily, we can use optical imaging to read out the calcium signals from those neurons for the same purpose.
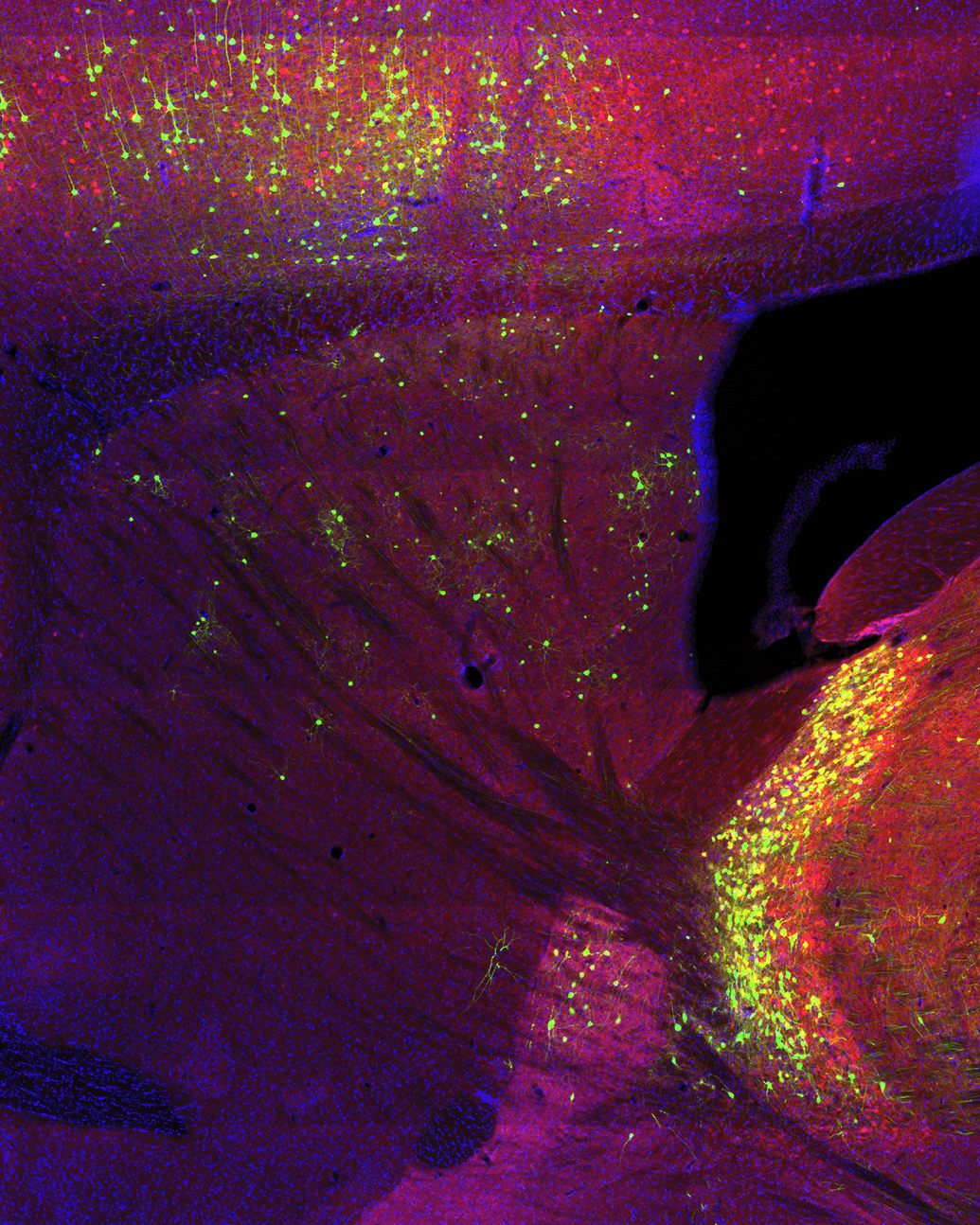
The third approach was the manipulation. This is crucial especially for studying motor systems because the motor system is highly redundant. For example, when you are driving a car, in 80% percent of the case you are not even aware that you are driving the car. You pay attention maybe 20% of the time. Even with the same outcome or consequence as it appears on the outside, from the inside, the behaviour might be controlled by completely different circuitries or mechanisms at the higher level. This is why it is both interesting and challenging to study the motor system – because we can achieve the same behaviour by very different means, and mediated by possibly different circuitries!
When we want to understand behaviour, we perform manipulations using advanced tools, which in our case is a lot of optogenetics because it is both temporally precise and targets specific cell types. In our study we tried to do everything closed-loop, meaning the manipulation was always based on the animal’s internal state or behaviour. The brain is only passively receiving the stimulation because as you can imagine, the same stimulation is going to trigger different behaviours if the brain of the animal were in different states. So the manipulation is always contingent on specific animal states or behaviour. That way, we can much better dissect that particular circuitry – what the contribution is – and get much more consistent results because we can better control for animal states.
With these three approaches to studying behaviour and physiology, including manipulation, we want to ask particular questions related to motor control. We can then hopefully transfer from mouse models to humans in clinical settings, through genetics and circuitry. In the end, we use manipulation to study behaviour and at the same time use mouse models to see if we can rescue certain aspects of disease. For example, if you activate the D2 pathway you can mediate switching behaviour. One obvious implication is that we can use D2-pathway-targeting drugs to potentially treat OCD or autism’s repetitive behaviours. That already points out a direction and target for treatment. That is one of the big goals of our work.
Were you surprised to find that there are specific neuronal populations in the cortico-basal ganglia circuitry that control the order and timing of actions?
Yes. We were surprised by several things. Firstly, we were surprised that different neuron types in the same brain region not only do different things, but also do things at a different hierarchical level. People expect from textbooks that there would be a quantitative difference. They think neurons will do something opposite from each other, but still on the same level – they add or reduce activity. But what we found is that there is some qualitative difference, and it controls different behavioural levels in the hierarchy. For example, in any organisation, especially in a university or institutional setting, you have the president, dean, director for each department, lab heads, then postdocs and students. What we were surprised to find is that actually, the group leader and dean are sitting in the same office, so to speak. People didn’t expect that.
Secondly, we didn’t expect the auditory system would be involved in timing. That was a big surprise for us and it took us a long time to confirm the results and figure out the neural mechanisms. And as soon as we figured that out in mice, it actually made perfect sense because I realised that we humans are doing this in a similar way. When I gave the task to the audience in the talk to open their eyes after they think 15 seconds have passed, the majority of the people likely counted by sound, either physically or in their head. It was surprising that we found this relationship between the auditory system and timing in mice, but also to realise that we use the same strategy as the mice.
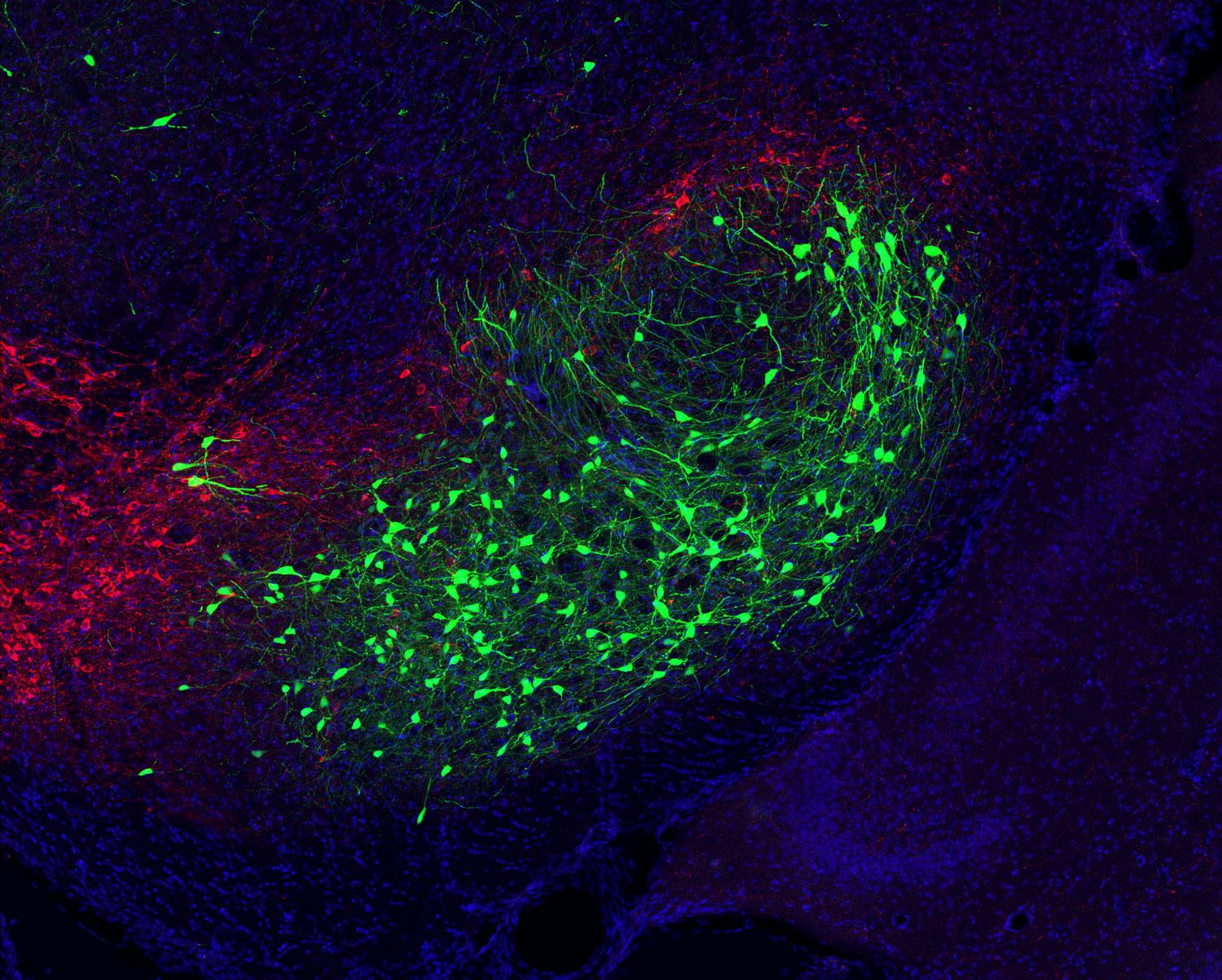
Thirdly, we were impressed by the degree of complexity in terms of the brain circuitry controlling the behaviour. Previously, we knew that the behaviour is complicated – as are human beings. We do things that we don’t sometimes even understand ourselves. But now, what we realise is that the brain is complicated not because there are so many neurons, but as our study shows, because the brain is organised in a very dynamic and hierarchical way to control the external hierarchy of behaviour. That level of complexity is impressive. We tend to think that one brain region is doing one major thing. But the reality is much more complicated, meaning we as neuroscientists have a much more difficult task to accomplish in future.
What implications do your research findings have for neurological diseases impaired in action sequencing in humans?
I’ll give you three examples. The first example is Parkinson’s disease. People with Parkinson’s have problems not only with initiation, but also with termination and switching. They fundamentally have a problem with changing from one state to another. Once they start moving, they cannot stop and once they stop, they cannot start moving. If they go in one direction, they cannot go in another direction. Parkinson’s is cause by the degeneration of dopamine neurons and dysfunction of the basal ganglia. That is tightly related to the new model and framework that we propose, that the indirect D2 basal ganglia pathway is crucial for switching behaviour.
The second example is OCD or autism. Kids with autism have a problem with repetitive physical behaviour – they keep doing the same physical motor action again and again. People with OCD have problems with repetitive thoughts – even if their hands are clean, for example, they keep thinking again and again they need to wash them. So if you go back to the framework I mentioned, we think the motor act and cognitive act share the same principle. The fundamental problem is that they have difficulty switching or transitioning from one action or thought to another.
And then you realise that this is the same in Parkinson’s! In Parkinson’s, there is a problem with switching or changing. In OCD or autism, there is the same problem of changing or switching. Previously, people have thought about these conditions in isolation from each other. For Parkinson’s people just thought the patients move less, or for OCD or autism people thought the patients act too much. But it turns out they might share the same problem at certain level. So that is why I think it’s very interesting – we didn’t think about this until we did all those experiments and came to the conclusion that actually, many neurological and psychiatric diseases have behaviours that may look very different, or opposite to one another, but the internal cause at the computational level is the same. How amazing is that? They share the similar problem, but the phenotypes look opposite.
These are three diseases that people usually group into two groups. One has little action or movement, and the other has too much. But in our study we try to link them together and point out they have the same problem of transition and switching. Put it more simply, they have the same problem of ordering actions. They cannot perform the action sequence in the proper order.
The third and final example is related to timing. We study timing because it’s inherently interesting to think about how the brain regulates time, but also because of its clinical implications.
The easy side of the coin to explain is that we all know, if you’re bored by a talk, the time slows down. You wonder why it feels so long! But if you are with someone you are happy with, or your friends or family with whom you get along, you feel like time flies. You ask why the holiday felt so short. And relatedly, on the clinical side, if someone drinks alcohol, time perception can be different when drunk. Time feels to pass more quickly. To go even further, if someone is high on a particular drug, cocaine or amphetamine, their physical movement is quicker and feels like time passes more quickly.
This shows that time perception is not a constant. It’s like Einstein’s theory of relativity – time is not constant as in the Newtonian framework, rather, it can be affected by the internal state, drugs, or aging etc. When we are young, time feels to pass slowly, but when we get older, years feel like months. It is quite interesting from this perspective, which is one side of the coin.
The other side of the coin is that in schizophrenia patients, for example, one symptom in some of the patients is that they sometimes think they were kidnapped by aliens. Since timing is critical for logic and causality, one possible explanation is that the dysfunctional timing function might underlie some of these symptoms. Logic relies on both timing and order of external events and internal actions. Only the things that happened before can cause the things to happen later. If this is messed up in the brain, and one have to be as rational as possible to communicate, sometimes an alien agency is needed.
What’s the next piece of the puzzle your research is focusing on?
There are three pieces. Firstly, we revealed just the tip of the iceberg in terms of the hierarchical control of behaviour. We have a very elegant behavioural task and already revealed the use of basal ganglia as an entry point for understanding how the D1 and D2 pathways control the hierarchy. And now, many follow-up questions can be asked, which involve knowing the upstream and downstream neurons. What information do upstream areas receive to make this decision? How does the brain decide to switch tasks? And how do downstream areas execute the switch? These questions are what we are working on right now, focusing on the communication between the basal ganglia and other brain regions. How do they work together to control the hierarchy of the behaviour?
Secondly, we are still trying to figure out the timing mechanism and circuitry. We propose a new theory about how the brain mediates timing, which is that it is fundamentally a sensorimotor integration mechanism.
The brain can automatically, consciously or not, track the environment and the animal’s own behaviour, then use that as a foundation to estimate and track time. The auditory cortex-to-basal ganglia pathway is important, therefore, to control the action. But in future we want to understand how the computation, or how each component of the clock, works. You have the pacemaker, which is the oscillator – every clock has an oscillating component, something changing. Then there is something driving the change. There is also a component that makes a comparison so that you know what it is. So we already figured out what is changing, which is the animal’s own behaviour, and the consequence of that behaviour. Now, we are trying to figure what is accumulated to measure that change. Which brain region is responsible? And how does this mechanism compare what it measures to memory? Every timing interval is learned. One minute does not mean anything to a new-born. We know how long one minute is based on our experience. So the three components we want to clarify are the oscillator, measurer, and memory.
Thirdly, in addition to the above two pieces of the puzzle that we have been working on for the past ten years, the major reason I moved back to Shanghai from the United States to work in East China Normal University and NYU Shanghai, is to do more research related to human patients. Not only can we study human patients in Shanghai thanks to a large volume of clinical hospitals we can work with, but more importantly we can use non-human primates (NHPs) as a bridge. From mice to the human, it’s possible to make a connection between their genetics, but we all know that their brain circuitries can be very different.
So I hope in the next five to ten years in the new lab at Shanghai to follow up on the similar action control questions as before, but move part of the effort to NHPs. The idea was to bridge the neural computation or mechanism from mice to humans, but use monkeys as a bridge. That is where you can actually dissect the circuitry and neural network activity. At the same time, we hope to move as treatments in humans from the insights of all the manipulation or rescue experiments that we performed on mice. But before we do that, we need to develop, test and optimize the techniques in NHPs. That is the final goal, which is to understand not only how the action is organised in the normal brain, but also to fix the problem of action control in human patients.
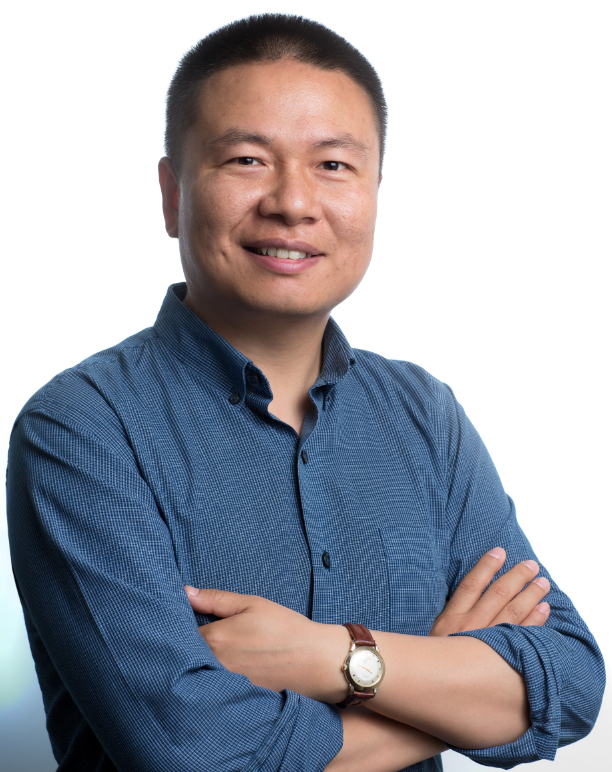
About Dr Xin Jin
Dr Xin Jin is a professor and director of Center for Motor Control and Disease at East China Normal University (ECNU), Shanghai. He was an Assistant Professor (2012-2017) and Associate Professor (2018-2020) of the Salk Institute for Biological Studies before joining ECNU in 2021. His lab research focuses on the basal ganglia circuitry and action control. The work has been recognized by many international awards including Portuguese Society for Neuroscience Featured Article Award, NIH Benedict J. Latteri Memorial Award, Society for Neuroscience Gruber International Research Award, and Mcknight Memory and Cognitive Disorders Award.